Significancy
The Ras superfamily of small GTPases is responsible for the control of many important cellular processes. These proteins are switched into an active signaling state (“on” state) by GDP to GTP exchange, and return into an inactive “off” state by a GTPase reaction (Figure 1). The defined control of the GTP hydrolysis is of vital importance for the regulation of several signal transduction processes in the living cell. Of high importance for medicinal chemistry here is the major role of the small GTPase Ras in the cell growth-signaling pathway: Site-specific oncogenic mutations inhibit the GTPase reaction, which causes the loss of down regulation of the downstream growth signal. This contributes to uncontrolled cell growth, and results finally in the development of tumors. Common to all members of the Ras superfamily is the catalysis triphosphate hydrolysis by the G-domain: Compared to hydrolysis of GTP in water, the hydrolysis in Ras is accelerated by five orders of magnitude from 200 days to 30 min under physiological conditions. However, to control growth signals in the living cell, GTPase-activating proteins (GAPs,) accelerate catalysis by further five orders of magnitude from 30 minutes to 50 ms.
Main Goals
One of our major research topics is to elucidate in detail, how this remarkable catalysis is obtained. A detailed understanding of structure, dynamics and function of Ras proteins and their oncogenic mutants enables the targeted development of drugs that attack dysfunctional proteins in order to cure diseases. We want to decoded how small GTPases enzymatically catalyzes the GTP hydrolysis and find common catalysis motives. Therefore, we unraveled the structures of the important steps of the catalysis reaction mechanism with sub Ångström resolution.
The enzyme catalysis is mediated by sub-Ångström structural changes of the substrate induced by the protein environment. We have developed and established a method to decode the three dimensional structural information and structural details of spectroscopic data by a combination of FTIR measurements and biomolecular simulations. The time resolved FTIR difference spectroscopy is highly sensitive regarding changes in structure and charge distribution. In combination with biomolecular simulation, we can generate validated structural models with an accuracy of up to 0.01 Å. These structures are experimentally validated by the comparison of the calculated spectral features like vibrational modes, isotopic shifts, and difference spectra with experimental ones. Only this high resolution allows for a complete understanding of catalysis.
The long-term goal of the research field of small GTPases is to understand the effect of oncogenic mutations. Despite of detailed structural insights of ground state of the wild type and mutants the function of oncogenic mutations still remains elusive. It is believed that the mutants effect the transition states or the positioning of the nucleophilically attacking waters. These very rare and transient structures are hard to obtain experimentally and within biomolecular simulations. Therefore, we developed a novel method called Local Mode Analysis (LMA) for calculating IR spectra and assigning spectral IR-bands on the basis of movements of nuclei and partial charges from just a single QM/MM trajectory (Figure 5). Through LMA the decoding of IR spectra no longer requires several simulations or optimizations. The novel approach correlates the motions of atoms of a single simulation with the corresponding IR bands and provides direct access to the structural information encoded in IR spectra.
Beside the investigation on an atomistic level, is it essential to consider the cellular environment on a molecular level as well. Attenuated Total Reflectance (ATR)-FTIR difference spectroscopy provides a tool to analyze membrane bound proteins in a near-native environment. The structure and orientation of the proteins bound to the membrane are decoded from the measured order parameter by bimolecular simulations.
Destabilization of the educt state
We elucidated the contribution of Ras, Ras•GAP and the Mg2+ to the catalysis of GTP hydrolysis by combining biomolecular simulations and FTIR measurements. The conformation of GTP·Mg2+ is changed by Ras from a staggered conformation of the non-bridging oxygen atoms of β and γ-phosphates in water to an eclipsed conformation. The change is due to the change in triphosphate coordination of the magnesium ion from tridentate in water to bidentate forced by Ras. The intruding arginine finger of GAP additionally induces an eclipsed position of the β and α-phosphates.
Figure 1: Schematic sketch of the reaction mechanism of the GTP hydrolysis enzymatically catalyzed by the Ras•GAP complex. The depicted structural models of the substrates were decoded from FTIR spectroscopic measurement by biomolecular simulation of the substrates bound to the solvated protein under physiological conditions. The tremendous catalytical effect of Ras•GAP of 105 can be explained by destabilization of the educt state in direction of the transition state. The calculated conformational energy difference of the functional complex of the phosphate group and the bound to Mg2+ of 28 kJ/mol is comparable to the free energy of activation of 33 kJ/mol, which was experimentally measured by Koetting et. al.
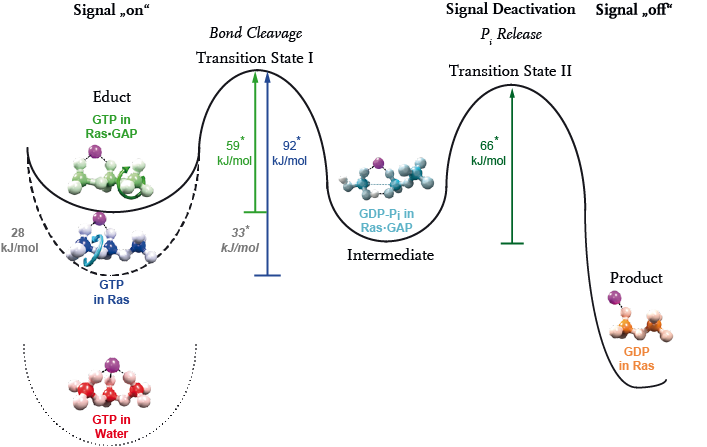
The conformational change of the non-bridging oxygen atoms from a staggered to an eclipsed position induces a lengthening of the bond between P3 and O32 that is cleaved during hydrolysis, with the γ-phosphate group becoming more planar. Concomitant changes in charge distribution facilitate the nucleophilic attack of a water molecule: the γ-phosphate becomes more positive, facilitating the positioning of the negatively charged oxygen of the attacking water, and two of the non bridging oxygen atoms of the γ-phosphate remain negative and are possible targets for proton transfer. All these effects already occur going from GTP•Mg2+ in water to Ras, and are enhanced by GAP. Further, the Mg2+ drags electrons from its surroundings before hydrolysis and stores them intermediately. The electron shift is reversed by bond cleavage and Pi release. The protein utilizes the tremendous effect of the magnesium ion by guiding it to a catalytically effective position.
In summary, these changes lower the barrier for hydrolysis by raising the conformational energy of GTP in the educt state and driving it closer to the transition state. The difference in conformational energies of the triphosphate magnesium ion moiety bound to Ras and Ras•GAP is about 28 kJ/mol, which is in excellent agreement with the energy necessary to accelerate hydrolysis by a factor of 105 (33 kJ/mol) in Ras•GAP as compared to intrinsic Ras. Obviously, part of the GTP binding energy is used to reduce the activation free energy barrier, which has to be overcome for hydrolysis.
We compared the GTPase reaction of the slower hydrolyzing GTPase Ran with our above described results for Ras. By combination of time-resolved FTIR difference spectroscopy and QM/MM simulations we elucidate that the Mg2+ coordination by the phosphate groups, which varies largely among the X-ray structures, is the same for Ran and Ras. This finding was confirmed by our collaborator at the MPI who obtained a new X-ray structure of a Ran•RanBD1 complex with improved resolution (PDB-ID 5CLL). The Mg2+ coordination is not responsible for the much slower GTPase reaction of Ran. Instead, the location of the Tyr-39 side chain of Ran between the γ-phosphate and Gln-69 prevents the optimal positioning of the attacking water molecule by the Gln-69 relative to the γ-phosphate. The steric hindrance through Tyr-39 is confirmed in the RanY39A•RanBD1 crystal structure. The QM/MM simulations provide IR spectra of the catalytic center, which agree very nicely with the experimental ones.
The combination of both methods can correlate spectra with structure at atomic detail. For example the FTIR difference spectra of RasA18T and RanT25A mutants show that spectral differences are mainly due to the hydrogen bond of Thr-25 to the γ phosphate in Ran. This work is a proof of principle for the high sensitivity of the integration of theoretical and experimental IR spectroscopy. Here, we have predicted IR spectroscopic shifts of mutations, which afterwards were verified by FTIR spectroscopic measurements. The difference of one hydrogen bond was resolved by both, theory and experiment. The integration of x-ray structure analysis, QM/MM simulations, and IR spectroscopy is a powerful tool to analyze the structure and dynamics of the catalytic center of a protein with atomic resolution. Only such atomic resolution provides the basis to understand protein catalysis.
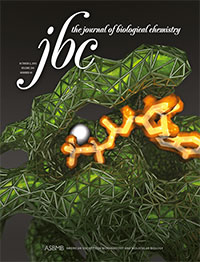
Figure 2. Summary of the main results from, x-ray crystallography, FTIR spectroscopy, and MD simulations. In a the comparison of the x-ray structures of RanWTGpp(NH)p-RanBD1 (PDB code 1RRP) (green) and RanY39A-Gpp(NH)p (PDB code 5CIW, dark green) is depicted. In RanWT Tyr-39 is fixed between the -phosphate and Gln-69. b, FTIR difference spectra of photolysis aph of RanWT-GTP-Mg2+-RanBD1 (light green), and RanY39A-GTP-Mg2+-RanBD1 (dark green). The intensity of the WT measurement is scaled by the factor 1.3. The shift of 12 cm-1 of the g-phosphate band reveals the hydrogen bond between Tyr-39 and g-phosphate, which is absent in RanY39A. c, comparison of the key residues in Ras and Ran involved in the steric hindrance as obtained from x-ray structures and the MD simulations. In Ran the oxygen of the Tyr side chain ismuchcloser to a line from the Gln C atom to the phosphor atom of the g-phosphate, leading to the hypothesis that the Tyr-39-OH hinders the Gln-69 side chain from assuming an optimum catalytic position close to the g-phosphate. This could be one of the explanations why the hydrolysis rate of Ran is slower that the one of Ras. All distances and the list of averaged PDB structures used to calculate the shown atom positions are given in supplemental Fig. S4. In d the averaged structure of the catalytic glutamine of the last 25 of 50-ns MD simulations of RanWT-GTP-RanBD1, RanY39A-GTP-RanBD1, RasWT-GTP, and RasY32A-GTP are represented. The colors display the simulated B-factor averaged over the side chain and backbone atoms. Note that the simulated B-factors are obtained from the fluctuation during theMDsimulation and are not directly comparable with the crystallographic B-factors.
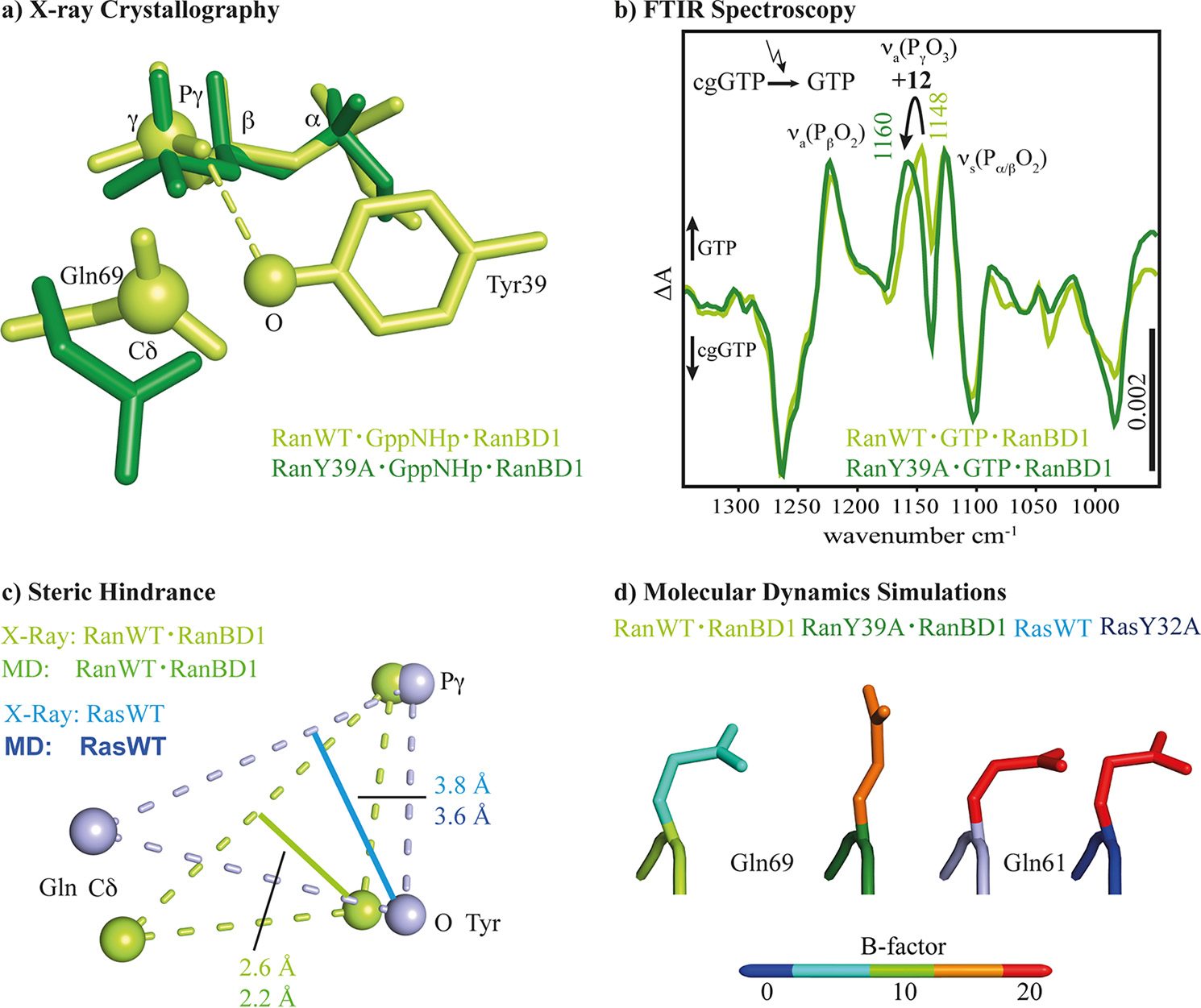
Beside the atomistic details, structural insights on a molecular level are also necessary. In order to elucidate the biological function of Ras in the cell, an investigation of the interaction and binding to the cell membrane via its lipid anchor is essential. The three most important Ras isoforms (N-Ras, K-Ras, and H-Ras) have their main differences in the lipid anchor region. This implies that the Ras isoforms are located at differently constituted parts of the cell membrane. Comparing the orientation of membrane bound full length N Ras relative to the membrane during MM simulations with order parameters obtained by ATR-FTIR spectroscopic measurements revealed that membrane bound N Ras forms an upright oriented dimer. This result was confirmed by FRET measurements, which are in good agreement with fluorophore distances of 46 Å predicted by biomolecular simulations. The computationally observed dimer interface can be investigated further by targeted mutagenesis studies. In addition, it clarifies results of cell biological studies, which reveal a contribution of residues predicted by us to be involved in the dimerization interface to the signal transduction.
Concluding, by our developed methods we have now access to experimentally validated structural and dynamical information on three levels: (1) functional chemical bond details with atomic resolution clearly below the resolution of X-ray structure analysis; (2) structure and incorporation of substrates in protein environment during transiently formed intermediates; (3) molecular structure and orientation of proteins bound to a membrane, including protein-membrane and protein-protein interaction. By these investigations, we gained a detailed understanding of the Ras catalysis mechanism. With our methods we provide powerful tools, which pioneer a new way of detailed structural analysis of molecular processes during enzyme catalyzes and transient ligand binding. Detailed structural insight is an important milestone within the long journey of small GTPase-targeting drug design for molecular cancer therapies.
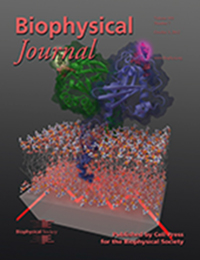
Publications
- Local Mode Analysis: Decoding IR spectra by visualizing molecular details. Massarczyk M.*, Rudack T.*, Schlitter J., Kuhne J., Koetting C., Gerwert K.
- Catalysis of GTP hydrolysis by small GTPases at atomic detail by integration of X-ray crystallography, experimental and theoretical IR spectroscopy. Rudack T.*, Jenrich S.*, Brucker S., Vetter I. R., Gerwert K., Kötting C.
- Ras and GTPase-activating protein (GAP) drive GTP into a precatalytic state as revealed by combining FTIR and biomolecular simulations. Rudack T., Xia F., Schlitter J, Kötting C., Gerwert K.
- Detailed Structure of the H2PO4 Guanosine Diphosphate Intermediate in RasGAP Decoded from FTIR Experiments by Biomolecular Simulations. Xia F.*, Rudack T.*, Cui Q., Kötting C., Gerwert K.
- The Role of Magnesium for Geometry and Charge in GTP Hydrolysis, Revealed by Quantum Mechanics/Molecular Mechanics Simulations. Rudack T., Xia F., Schlitter J., Kötting C., Gerwert K.
- N-Ras forms dimers at POPC membranes. Güldenhaupt J.*, Rudack, T.*, Bachler P., Mann D., Triola G., Waldmann H., Kötting C., Gerwert K.
- Exploring the Multidimensional Free Energy Surface of Phosphoester Hydrolysis with Constrained QM/MM Dynamics. Li W., Rudack T., Gerwert K., Gräter F., Schlitter J.
- The specific vibrational modes of GTP in solution and bound to Ras: a detailed theoretical analysis by QM/MM simulations. Xia F., Rudack T., Kötting C., Schlitter J., Gerwert K.
Journal Physical Chemistry B, Klaus Schulten Memorial Issue, in press
Proceedings of the National Academy of Science of the USA 2012 109:15295-15300.
Journal of the American Chemical Society 2012 136:20041-20044.
Biophysical Journal 2012 103:293-302.
Biophysical Journal 2012 103:1585-1593 (Cover).
Journal of Chemical Theory and Computation 2012 8 (10):3596-3604.
Physical Chemistry Chemical Physics 2011 13:21451-21460.
* equal contribution
Investigators
- Dr. Till Rudack
- Matthias Massarczyk
- Daniel Mann
- PD Dr. Juergen Schlitter
- PD Dr. Carsten Koetting
- Prof. Dr. Klaus Gerwert
Collaborators
Recent
- Shanghai Institutes for Biological Sciences (SIBS), Chinese Academy of Sciences-Max-Planck Partner Institute for Computational Biology (PICB), Shanghai, China
- Caroline Kammerlin, Uppsala University, Department of Cell and Molecular Biology, Uppsala Sweden
- Xia Fei, East China Normal University, Department of Chemistry, China